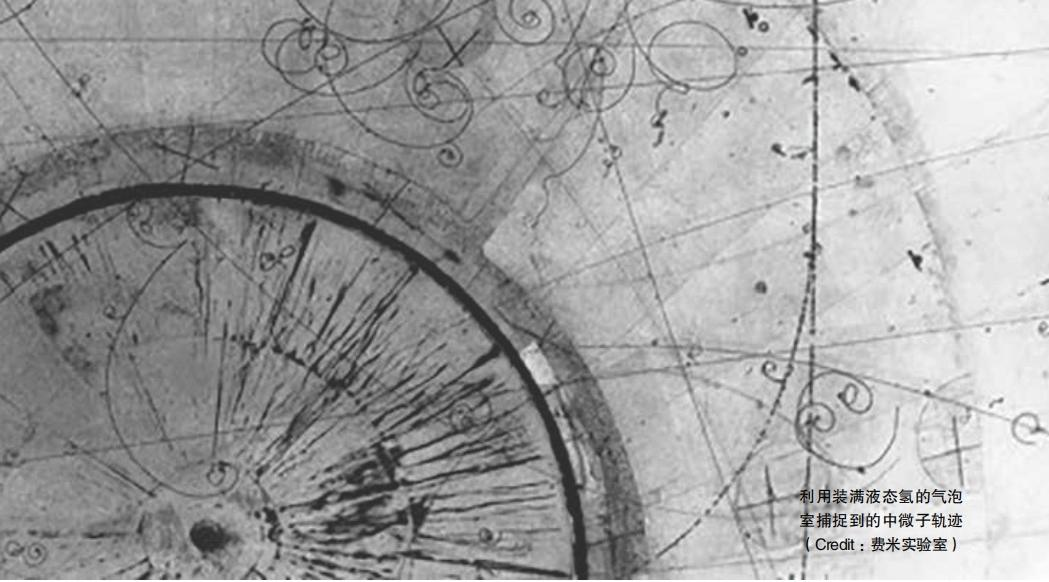
Zip! Zing! Right now, teeny tiny paricles are pelting your body. Every second, one hundred trillion of them zoom through you. But they don’t hur. In fact, they usually don’t disturb even a single atom or molecule on the way through. These paricles can pass through all of planet Earh without hitting anything!
What are these sneaky little things? They are called neutrinos. They come in three varieties, or flavors, called electron, muon, and tau. They have no electric charge, which is one way they difer from the electrons in atoms. Oddly, a neutrino switches between all three flavors as it travels, a trait called oscillation. Neutrino flavors have different masses that are all extremely close to zero. Neutrinos are “really, really, super light,” says Kate Scholberg. She’s a physicist at Duke University in Norh Carolina. An electron is the lightest paricle in an atom of typical matter. But neutrinos make them seem heavy. Scholberg says, “If an electron is an elephant, a neutrino is lighter than a mouse.”
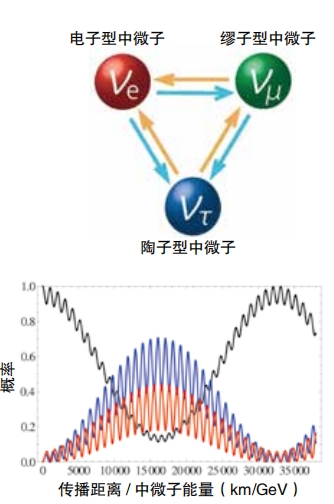
Almost all the neutrinos zipping through Earth come from the sun. But neutrinos come from all kinds of diferent sources, including black holes merging or a star exploding in a supernova. A banana even emits neutrinos–they pop out when potassium atoms go through radioactive decay. The standard cosmological model predicts that neutrinos left over from the big bang still pepper the universe.
Neutrinos are everwhere! There are far more of them in the universe than any other paricles.
The Ghost Paricle
Austrian physicist Wolfgang Pauli predicted that neutrinos should exist back in 1930, long before anyone detected one. A few years later in 1934, Italian-American physicist Enrico Fermi gave the particle its name. “Neutrino” means “little neutral one” in Italian.
These physicists came up with the idea of the neutrino to solve a problem. Energy seemed to go missing during beta decay, a process that happens inside some types of radioactive atoms. In one type of beta decay, called beta minus, a neutron turns into a proton and an electron. According to the law of conseration of energy, the energy of the electron should always equal the diference between the energies of the neutron and the proton. But when physicists measured these electrons, they had all sors of energies, and all of them were smaller than predicted. It seemed as if some of the energy had disappeared! Some physicists wondered if the law of conseration of energy didn’t apply to such tiny particles. But Pauli proposed that a new, electrically neutral paricle had carried away the missing energy.
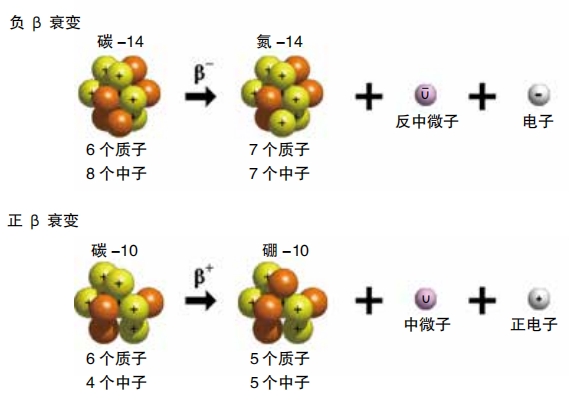
Physicists realized that neutrinos, if they existed, would be especially difcult to detect. Some even thought this would be impossible. “There is no practically possible way of obsering the neutrino,” wrote by nuclear physicist Hans Bethe and Rudolf Peierls in 1934.
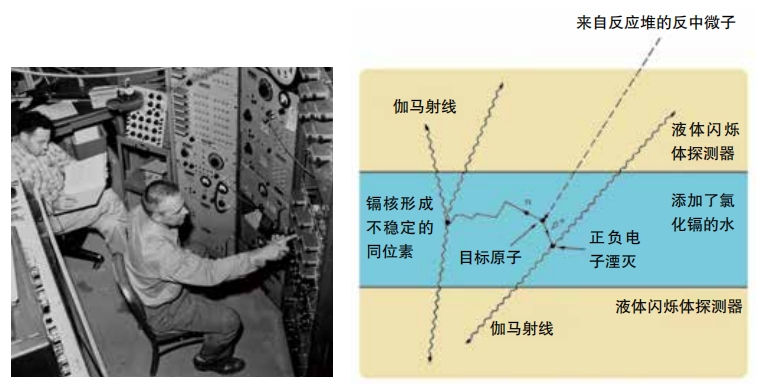
The problem was that a particle has to interact with something for us to be able to obsere it. You see things with your eyes, for example, because light hits the things you’re looking at. Scientific instruments can capture other kinds of evidence, such as scattered particles. Most of the time, though, neutrinos are essentially invisible—nothing hits them and they don’t hit anything, so it’s hard to obsere them. “They are like ghosts,” says Scholberg. In fact, a common nickname for the neutrino is “the ghost paricle”.
Ver rarely, though, a neutrino will happen to hit another particle. This is such a rare occurrence that in your entire lifetime, a neutrino will smack into an atom in your body just a handful of times. Could scientists fnd a way to catch this rare event happening?
In 1956, physicists Frederick Reines and Clyde Cowan were working on Project Poltergeist, an effort at Los Alamos National Laborator to catch neutrinos. The thing that fnally helped reveal neutrinos’ existence was a technology invented in 1942: the nuclear reactor, invented in 1942. A nuclear reactor gives off huge numbers of neutrinos. And the more neutrinos there are, the greater the chance that a few of them will hit something in a detector. Reines realized that setting up a neutrino detector in a nuclear power plant just might work. So that’s what he and Cowan did. In 1956, they sent a telegram to Pauli. It read, “We are happy to inform you that we have defnitively detected neutrinos.” Reines went on to earn the 1995 Nobel Prize in Physics for the discover.
A Cube beneathAntarctica
Today, scientists keep an eye out for neutrinos at around a dozen different observatories. These capture evidence of natural neutrinos produced in space. The largest neutrino obserator in the world, IceCube, began operating in 2011 in Antarctica. “It’s mind-bogglingly huge,” says Scholberg.
To build IceCube, engineers drilled eighty-six boreholes, and depths range from about 1.5 km to 2.5 km. These holes form a hexagonal pattern that’s about a kilometer across in length and width. Each borehole contains a string of spherical digital optical modules (DOMs) that can detect and amplify radiation signals. In total, IceCube has 5,160 DOMs, keeping watch for neutrino collisions, almost like security cameras. Neutrino collisions are very rare, and that’s why IceCube is so huge. The more space a neutrino detector watches, the greater the chance that it will catch some rare collisions. In its first twelve years of operation, IceCube caught detected hundreds of thousands of neutrinos—mostly coming from the Sun.
IceCube can’t see the neutrinos themselves. Instead, its sensors notice the mess a neutrino makes when it bashes into a molecule of ice. “The neutrino sor of splatters a nucleus of an atom and you get a whole bunch of paricles coming out in a big shower—that’s what we can see,” explains Scholberg.
Normally, nothing can move faster than light. But light itself slows down as it moves through ice and some other materials. The paricles that burst out after a neutrino collision move more quickly than light does inside ice. This causes something called Cherenkov radiation. “It’s kind of like a shock wave of light,” says Scholberg. And seeing it in the IceCube detector is a sure sign that a neutrino passed through. The pattern of the Cherenkov radiation reveals the direction a neutrino came from, its energy, and sometimes what favor it was.
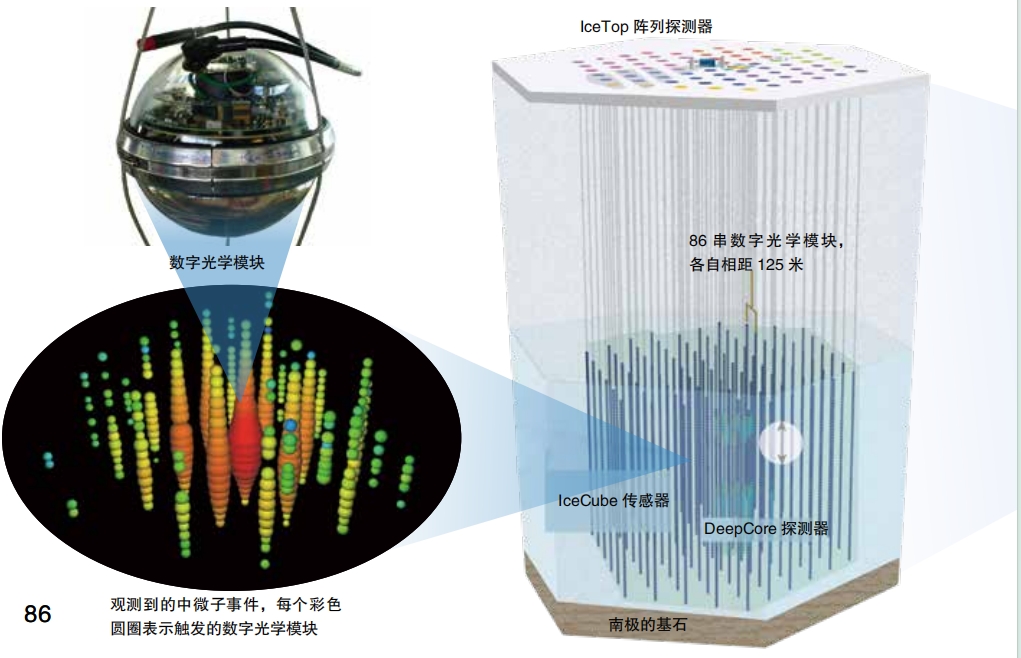
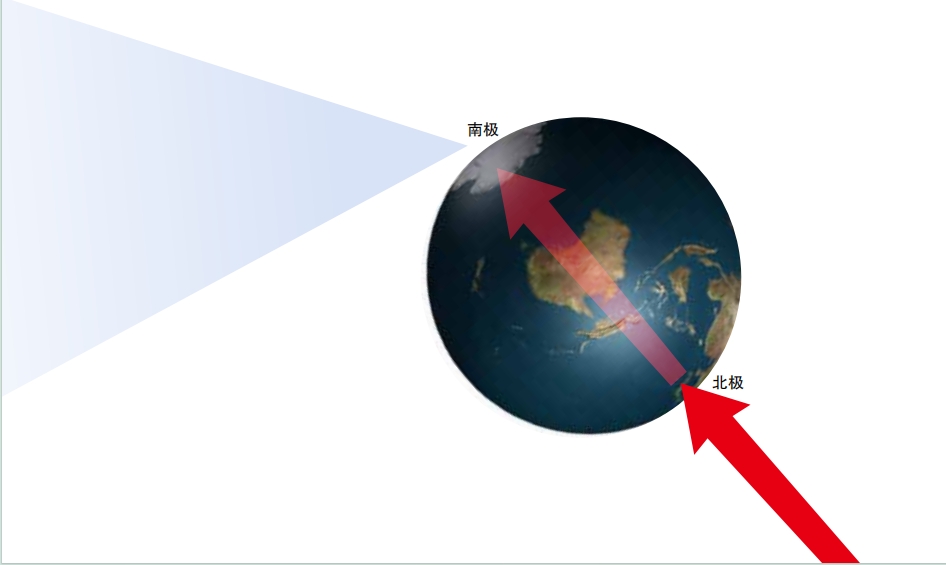
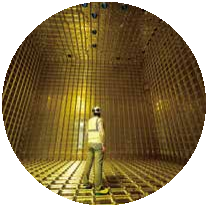
Interestingly, IceCube and other neutrino detectors don’t always look up toward the sky. They can also look through the center of the Earh, toward space on the other side. That’s because most neutrinos zip through the bulk of Earh all the way to the detector. Since most of the neutrinos a detector catches come from the sun, a neutrino detector can make a picture of the sun from underground.
When something exciting happens out in space, like two black holes merging or a star exploding in a supernova, a huge burst of neutrinos streams outward. When the neutrinos from one of these events pass through Earh, they make the entire ice glow for a few seconds. The glow isn’t visible to human eyes, but the sensors pick it up. Neutrino obseratories wait and watch for these special events.
Neutrinos can act as an early warning system for a supernova. That’s because they begin streaming outward from such an event before light does. Supernovas occur in the Milky Way about once every ten to fifty years. In 1987,supernova SN1987A triggered three separate neutrino detectors.
Scientists have plans for several exciting new neutrino observatories. To build a detector called the Deep Underground Neutrino Experiment (DUNE), engineers are excavating an area the size of eight soccer fields around two kilometers below the surface of South Dakota in the US. When DUNE is complete, those caverns will contain four tanks of liquid argon, each the size of a seven-stor building.
Another neutrino detector, the Pacific Ocean Neutrino Experiment (P-ONE) isn’t under construction yet. Scientists hope to build it at the bottom of the Pacifc Ocean. Strings of detectors anchored to the sea foor will reach upward, swaying in the water like strands of kelp and watching for neutrino collisions. China is planning a similar detector named TRopIcal DEep-sea Neutrino Telescope (TRIDENT) that would be anchored at the bottom of the South China Sea.
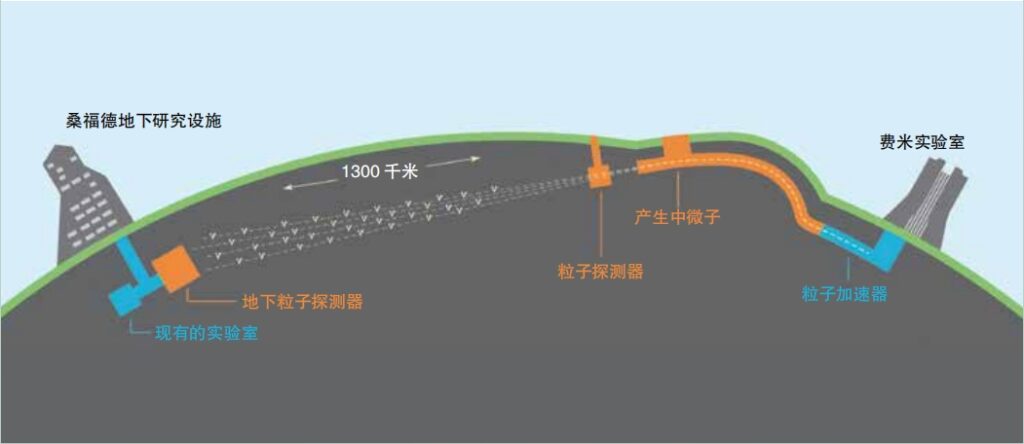
The Most Ghostly of All
IceCube and all the other neutrino detectors people have built or plan to build can only capture high-energy neutrinos. These neutrinos move at close to the speed of light. However, not all neutrinos are that speedy. Over time, a neutrino slows down and loses energy. “The lower the energy of the neutrino, the less likely it is to interact. And they’re already practically not interacting at all,” says Scholberg.
Physicists don’t know how to build a detector that might have a chance of catching these most-ghostly of all ghost particles. But they are working hard to figure out how this might be done. That’s because the most sluggish, low-energy neutrinos of all are especially important to our understanding of the universe. These neutrinos, the cosmic neutrino background (CNB), are the ones produced during the universe’s birh in the big bang.
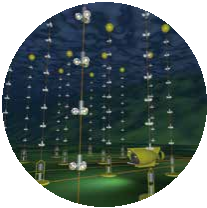
The neutrinos that obseratories can capture have energy in the mega-electron-volt range or higher. Cosmic neutrinos are predicted to have a wimpy 168 micro-electron-volts of energy. That’s billions of times less energy, and billions of times less likelihood of interacting with anything. These neutrinos should be spread out evenly all over the universe, with more than 300 cosmic neutrinos in ever cubic centimeter, a space around the size of the tip of a fnger. Detecting them directly is “the holy grail of neutrino physics,”. It’s a goal everone hopes to achieve someday.
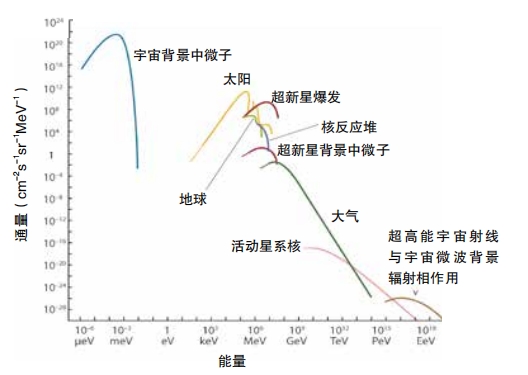
Scientists have already detected the cosmic microwave background, which is light left over from the birh of the universe. However, The photon didn’t unbound and star shining until the universe was already around 379,000 years old.
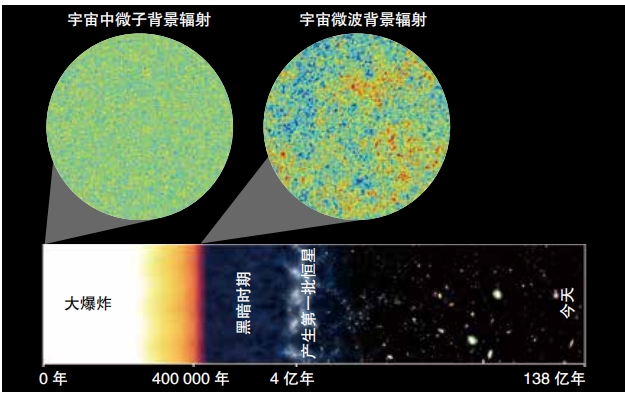
According to the standard cosmological model, the newborn universe had an extraordinarily high temperature and density, so all paricles collided frequently—even neutrinos. As the universe’s temperature cooled, it began expanding rapidly, so density dropped suddenly. This happened about one second after the universe’s birh. At this point, most paricles were still colliding a lot because of electromagnetism and the strong nuclear force. But neutrinos only feel the weak nuclear force, which wasn’t strong enough to keep them trapped. So they have been foating freely everwhere in the universe ever since. This is the cosmic neutrino background.
If scientists could measure the CNB, it could reveal whether the standard cosmological model’s version of the universe’s birh is correct or needs some rethinking.
Mysterious Mass
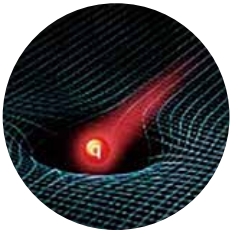
paricles, like electrons and protons, interact with something called the Higgs field. This field prevents them from moving at the speed of light, slowing them down and giving them a feature we call mass. The more a particle interacts with the field, the slower it goes and the more massive it is. But neutrinos don’t seem to interact with this feld at all. So they shouldn’t have any mass. But they clearly do. So where does it come from? Physicists have yet to solve this myster. But they are narrowing down what the diferent neutrino masses might be.
In 2024, researchers working on the KATRIN experiment, located at the Karlsruhe Institute of Technology in Germany, announced an upper limit on the masses of neutrinos. The paricles must be lighter than 0.8 eV/c2. “Hopefully a positive neutrino mass measurement is just around the corner,” said Joseph Formaggio, a physicist at MIT in Cambridge, Massachusetts, who is par of KATRIN.
Neutrinos may be super-light. But since they have mass, that means they contribute to the total mass of the universe. The standard model of cosmology says that the universe contains 5 percent ordinar matter, 27 percent dark matter, and 68 percent dark energy.
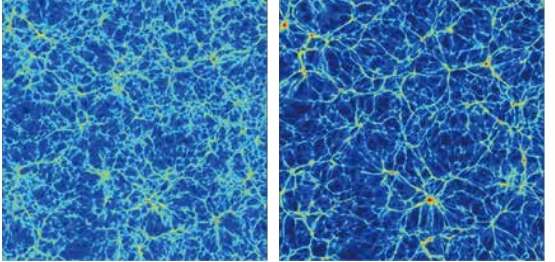
Physicists don’t know what dark matter might be made of. High-energy neutrinos, like the ones coming from the sun or a supernova, are too energetic to make up dark matter, though some like to call them a type of “hot” dark matter. Slow-moving neutrinos, like the ones in the CNB, are considered part of the dark matter, says Scholberg. Most likely, they make up around 0.5 percent to 1.5 percent of the universe’s dark matter. Knowing neutrinos’ masses more precisely would help cosmologists better understand how the universe and its galaxies formed and changed over time.
Early on in the formation of the universe, neutrinos likely kept matter from clumping up too tightly. That’s because these ghostly particles could zip through clumps without getting stuck. And sometimes their mass may have been enough to pull bits of matter away from clumps. This could have helped defne the locations where galaxies, stars, and other structures began to form.
Why Does Matter Exist?
Neutrinos may hold the answer to yet another big unsolved myster: why is there something instead of nothing? Every kind of particle has a twin called an antiparticle. It has the same mass but opposite electrical and magnetic properies. If a paricle and its antiparicle meet, they destroy each other. They disappear and become pure energy. According to the standard cosmological model, the big bang created equal amounts of matter and antimatter. It seems that these should have all destroyed each other. If that had happened, “the universe would be void,” Stefan Schönert told Quanta magazine. “It would be ver, ver boring for us, who would not exist.” He is a physicist at the Technical University of Munich and par of the GERDA Collaboration.
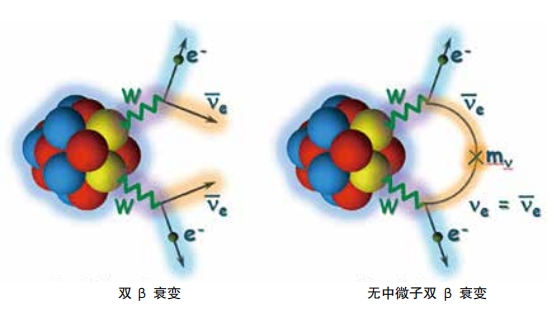
Since we’re here now, though, we know matter somehow got the upper hand and became dominant. This is an asymmetry that physicists would love to explain. And they have some ideas that involve neutrinos.
One theory holds that in the very early universe, there may have been some very heavy neutrinos hanging around. Some of these neutrinos would have decayed in a cascade of reactions. And this process would eventually produce slightly more matter than anti-matter. (Some heavy neutrinos may still be hanging around, and could account for more of the mysterious dark matter.) For a neutrino to decay in this way, it likely would have to be its own antiparticle. That means it could be observed as either a neutrino or an antineutrino, oscillating between the two states. Is this actually what happens? Physicists don’t know. “The answer to that question is a huge deal,” says Scholberg.
The GERDACollaboration is looking for an answer. They are hoping to observe an extremely rare natural process in which two neutrons in the nucleus of an atom decay at the same time. The theor is that they could become two protons and emit two electrons, but no neutrinos. This is called neutrinoless double-beta decay. It can only happen if neutrinos and antineutrinos are really the same kind of paricle.
Interestingly, this could also explain the other puzzle—why neutrinos have mass. That’s because the neutrino/antineutrino particle flip-flop their quantum state maybe decide their mass.
Physicists may have caught ghost paricles. But they have yet to fully understand their mysterious nature. What they learn may lead to a new understanding of our universe.